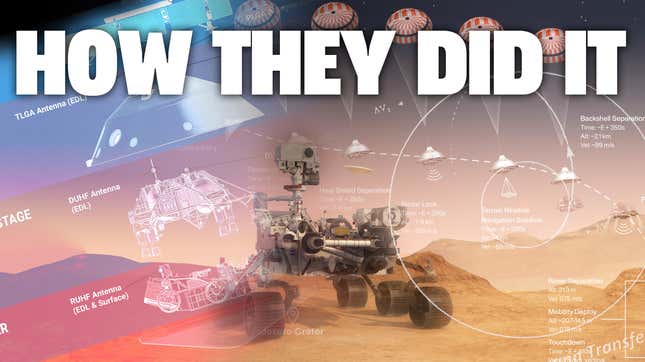
The world rightfully gawked at the engineering prowess of NASA’s Jet Propulsion Laboratory as it successfully landed the car-sized rover named Perseverance on the surface of Mars. You might think that I, an engineer with experience working on a deep space mission, would be less impressed than most. But trust me: The spacecraft that landed Perseverance was an incredible Rube Goldberg Machine, and I’m going to explain exactly how incredible in this in-depth technical breakdown, which features testing footage, as well as NASA’s newly released video and pictures from the landing and its aftermath.
The Mars 2020 Mission has been hailed as one of the most accurate and complicated pieces of human exploration ever attempted. It traveled almost 300 million miles from Earth to hit a bullseye less than five miles in diameter on the surface of Mars.
I’ve spent hours poring over the technical details of this feat, and everywhere I look, it becomes more elegant, complex, and impressive. Let’s dig into why the team needed this level of landing precision, how the spacecraft got to Mars, and how the craft descended to the surface. Along the way, let’s look at the testing and technical teamwork needed to hit that bullseye while decelerating the rover from 12,000 mph at the top of the atmosphere to less than two mph at touchdown, all in less than seven minutes.
A Small Landing Target Filled with Danger
The Mars 2020 Mission is looking for life that may have existed on the surface of the planet billions of years ago. The mission is first seeking to identify areas on Mars where previously-existing environmental conditions could possibly have supported microbial life. If NASA finds such an area, the team will scour it for the remnants of ancient life using the rover’s onboard instruments. The rover is capable of drilling and caching samples to be returned to Earth by a future mission.
The Jezero Crater: A Promising Spot That Could Once Have Facilitated Life
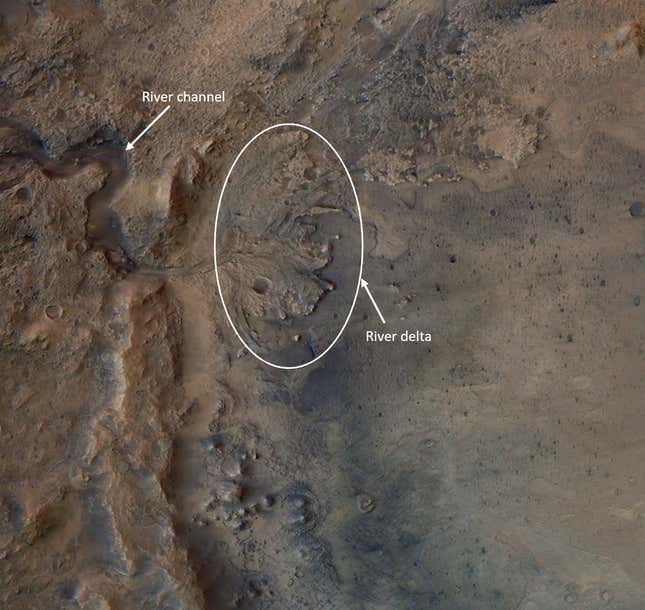
To set themselves up for success, scientists needed to pick a landing site within one of these areas thought to have once contained life-facilitating environmental conditions, and they found that site in Jezero Crater (pictured above). It is believed that this crater is a dried-up lake bed of an ancient body of water that was once about the size of Lake Tahoe. Specifically exciting to scientists is the fact that remnants of a river channel, and what appears to be a river delta, can be seen from orbit.
River deltas are made when water picks up material (mud, sand, etc.) from upstream and then drops that material off as the water slows when entering into a large body of water. This upstream material is deposited and preserved in the river delta, allowing scientists the opportunity to study the environments that existed upstream without actually having to drive the rover there.
Another interesting thing about Jezero Crater is that spacecraft orbiting mars have detected carbonate molecules in the area. Carbonate molecules are made when carbon dioxide reacts with water and rocks. This is exciting because, on Earth, carbonate molecules that precipitate out of the water in lakes and oceans are known to trap evidence of microbial life. Perhaps the same could have happened on Mars.
If you want to learn more about why Jezero Crater is so interesting to scientists, watch the video below to hear one of the science team members, Briony Horgan, discuss Jezero and its similarities to a lake in Turkey:
In this other video you can get more familiar with the topography of Jezero via a 3D fly over:
The Jezero Crater: A Scary Spot for Landing a Rover
While all that geology truly makes Jezero a great place to explore scientifically, to the engineers in charge of landing the rover on the surface it means something else entirely. At a press conference before the landing attempt, the Entry, Descent, and Landing (EDL) Lead, Al Chen, said, “Jezero Crater is a great place, a magnificent place, for science, but when I look at it from a landing perspective, I see danger.”
“It’s a formidable challenge,” he went on. “The site is replete with steep cliff sides that happen to run right through the middle of the landing site, there’s sand, there’s boulders, there’s impact craters — all these would be a bad day if we touched down on them.”
In fact, when the team analyzed the crater, the group realized that it couldn’t find a precise landing site near the river delta that was both within the team’s targeting capability and also entirely devoid of dangerous obstacles. So the engineers decided to make a map of safe places to land, and resolved to develop the technology needed to get there.
The images below show the contrast between how the science team sees the landing area and how the Entry, Descent and Landing Team views it. The image on the left is a spectral image taken from the Mars Reconnaissance Orbiter (Note: This is not real color); it shows the carbonate molecules that may contain trapped ancient microbial life in green. On the right is the EDL team’s avoidance map. In this map, the black ellipse is the landing target (4.8 miles x 4.1 miles), and the red areas indicate the hazards to be avoided for a successful landing.
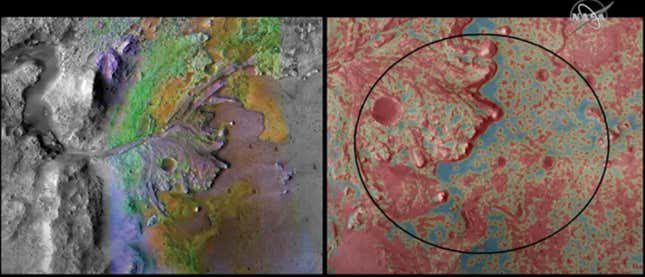
That’s a lot of red.
When explaining the hazard map, EDL systems engineer Erisa Stilley said, “For EDL we ask the question: ‘What could kill us on landing day?’ In the southeast, are rocks that are strewn throughout the Jezero Crater landing sight, in the southwest and to the north, we have sand dunes, and then that beautiful river delta we keep talking about, well, that looks like a 250 ft cliff to us. We certainly don’t want to land on that.”
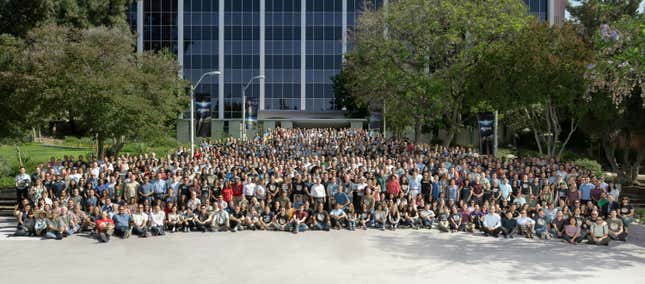
When I look at that map and think about the science questions that might be answered as a result of the rover landing successfully, and that the work of so many people hung in the balance, I can’t help but think that this was the most intense game of ‘the floor is lava’ that I’ve ever seen.
An Energy Management Problem
Lots of Kinetic and Potential Energy To Scrub Off
It’s not just the small landing zone riddled with hazards that made this whole endeavor difficult, however. It’s also the fact that the spacecraft arrived at Mars with tremendous velocity, and that speed had to be reduced in just such a way that when the rover arrived at the landing spot, the six-wheeled machine’s velocity was low enough to safely touch down without damage.
The best way to appreciate how the team achieved its goal is not to focus too much on the speed part of the equation, but rather to consider the total energy of the system.
See, this is an energy management problem. The goal was to slow the rover down in just such a way that its energy reached essentially zero precisely when the spacecraft reached the designated landing spot, and to do this in an efficient manner without damaging the rover. As a refresher, let’s talk about two forms of energy that are involved in this problem: kinetic and potential energy.

Kinetic energy is the energy an object has by being in motion. It’s proportional to the mass (m) of the object and the square of its velocity (v). Said another way, if you halve an object’s mass, you also halve its kinetic energy, and if you double it’s velocity, you quadruple its kinetic energy.
In this case, potential energy is the energy the spacecraft has due to its position relative to Mars. Since the spacecraft was accelerating due to the pull of Mars’ gravity, there was a ‘potential’ for the spacecraft to gain kinetic energy as it got closer to Mars. Potential energy is proportional to the mass of the vehicle (m), the altitude above the surface of Mars (h), and the gravitational acceleration of Mars (g, about a third of that here on Earth).
In the case of landing Perseverance on Mars, potential energy was either converted into kinetic energy by reducing the spacecraft’s altitude or it was reduced by removing some of the spacecraft’s mass.
At the top of Mars’ atmosphere, roughly 83 miles above the planet’s surface, the spacecraft was traveling about 12,000 mph. This means the spacecraft had a kinetic energy of 44 gigajoules and a potential energy of 1.2 gigajoules. To give you an understanding of the total energy we’re talking about, this is about the same amount of energy released by the MOAB bomb, one of the largest non-nuclear bombs in existence:
The Anatomy of the Mars 2020 Spacecraft
To solve this energy management problem and get the fast-moving, high-flying spacecraft to reach the surface at a very low velocity, the team designed and tested an immensely complicated machine, pictured below in its five main components. From top to bottom, the vehicle consists of 1) The cruise stage – responsible for getting the rest of the spacecraft to Mars 2) The backshell – the purpose of this is to protect the rover while it descends through the atmosphere. It also helps to steer to the landing site during atmospheric entry, and contains the parachute 3) The descent stage – This is a jetpack for the rover that is used to slow the rover during the final part of descent, and lowers it to the surface like Tom Cruise in Mission Impossible 4) The Perseverance Rover – the car sized robot that actually does the science exploration once on the surface 5) The heatshield – the sacrificial cover that works with the backshell to protect the rover while the spacecraft slams into the Martian atmosphere.
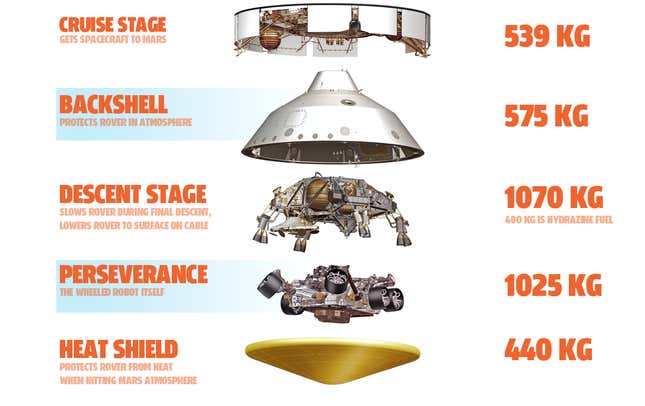
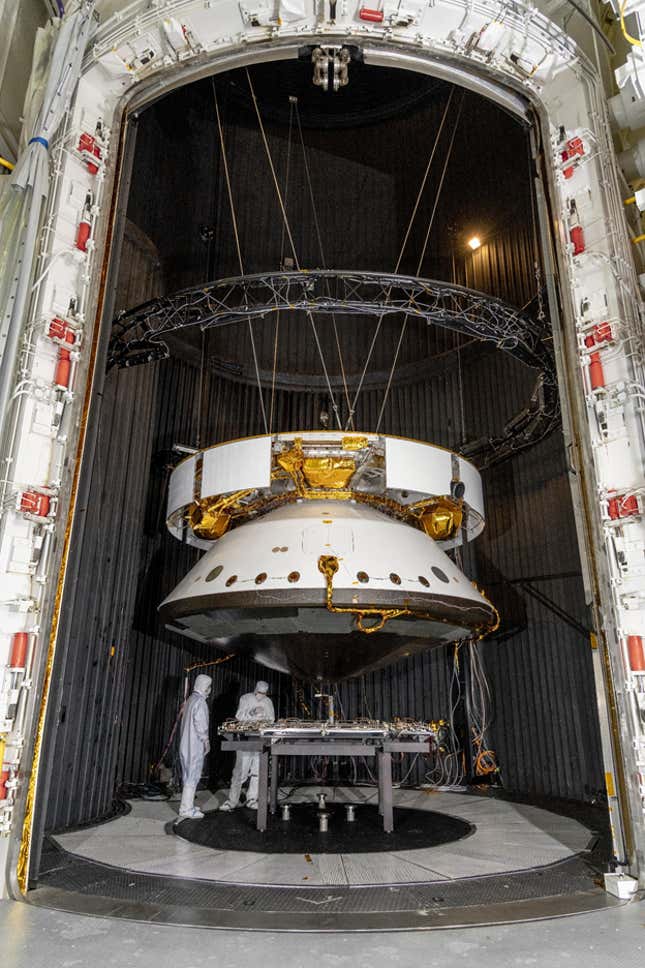
Below you’ll find an image outlining the general steps of how the team solved the problem, along with a video explanation. Both of these provide a high-level overview of the landing sequence, and in general, this is about as detailed as most write-ups on the landing get. But this isn’t like most write-ups; we’re going to take a closer look.
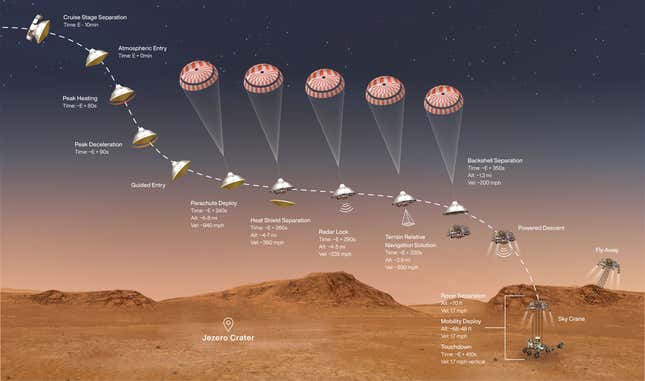
Hitting a Bullseye 300 Million Miles Away
To even make an attempt at landing, the team first had to not just get the rover near Mars, but deliver the vehicle to a precise point at the top of the Martian atmosphere at a precise time. This was done to limit the scope of the energy management problem. By delivering the vehicle with high precision, the team knew the starting energy the vehicle would need to burn off before landing, and the brilliant engineers understood over how much distance that energy had to be scrubbed off. Narrowing the range of potential starting conditions made the difficult problem of landing just a little easier to solve.
So how’d the team accomplish this delivery, and how precise was it?
The Hohmann Transfer Orbit: Establishing the Route To Mars
The mission launched from Cape Canaveral on an Atlas V-541 launch vehicle on July 30th, 2020, breaking free of the earth’s gravity with a speed in excess of 24,000 mph relative to the earth.
The launch put the Mars 2020 spacecraft on what’s called a Hohmann Transfer orbit—a maneuver that is specifically designed to use the least amount of fuel when transferring from one orbit to another. In this case, the transfer orbit was designed to go from the earth’s heliocentric (sun-centered) orbit to Mars’ heliocentric orbit, and was designed to take advantage of an alignment that occurs about every 26 months between Earth and Mars. In the picture below, you can see an illustration of the transfer orbit, with the spacecraft traversing the solar system starting from earth’s location on launch day and then aiming for where Mars would be on landing day.
The launch vehicle itself was not precise enough to ensure the spacecraft arrived at the aim point, so the engineering team designed in the capability to track the spacecraft’s transfer orbit, and to make adjustments to its trajectory so that it would arrive precisely when and where the team was aiming. These opportunities to make the adjustments to the orbit are called Trajectory Correction Maneuvers or TCMs, and the team planned to use up to six of them.
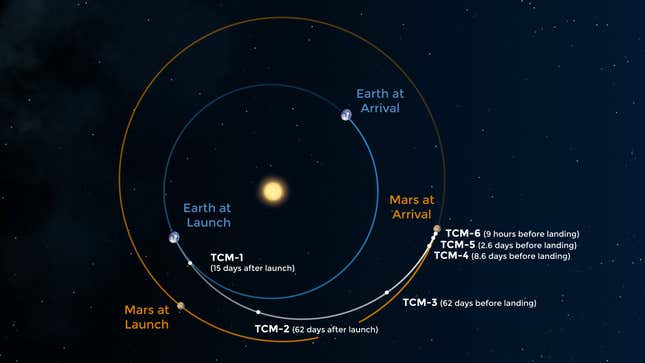
Each of these TCMs required a significant amount of coordination between multiple teams, a wide and deep technical knowledge and skill set, and the use of precise technology both on the spacecraft and here on Earth.
To pull maneuvers like this off, the team first had to understand the spacecraft’s location and velocity. Engineers used that information to assess if the spacecraft would arrive at Mars at the right time and location. When not on target, the team commanded the spacecraft to execute a maneuver using the cruise stage’s thrusters to get back on target.
Determining the Spacecraft’s Position and Velocity
The Mars 2020 mission uses the Deep Space Network, a group of large ground based antennas placed around the world, to monitor telemetry (data) from the spacecraft and to send it commands. By analyzing the radio signals sent between the ground antennas and the antennas on the spacecraft, the team was able to determine the spacecraft’s position and velocity via three techniques called ranging, delta-DOR (differential one-way ranging), and Doppler.
Ranging is a technique used to determine the distance between the spacecraft and the ground-based antenna. The range of the spacecraft is determined by measuring the time it takes for a signal sent from the ground antenna to the spacecraft and vise versa. By measuring the time it takes for these signals to travel and by knowing the speed of light, engineers can determine the distance, or the range, to the spacecraft.
Delta-DOR, is used to determine the spacecraft’s position in the earth’s sky and builds upon the standard ranging technique. In this technique however, two ground based antennas on opposites sides of the earth listen for signals from the spacecraft at the same time. By understanding the differences in times the signal from the spacecraft arrives at one ground based antenna verses the other, the angular position (where to look in the sky to see the vehicle) of the spacecraft could be determined.
Doppler is a technique used to determine the velocity (the speed and direction of the movement) of the spacecraft by measuring the frequency of the radio signals received by the earth antennas and the spacecraft. The team analyzes the frequencies to determine the velocity by understanding the Doppler Effect. Similar to the change in pitch you hear as a fire truck drives by you (higher pitch if coming toward you and lower if going away from you), the radio frequency received by the spacecraft or the ground antenna will differ from the frequency it was sent at based on the velocity of the spacecraft.
Using the range, angular position, and velocity of the spacecraft, engineers were able to model the spacecraft’s trajectory (where it was and where it was going). Most importantly, the team could determine if a trajectory correction maneuver was required so that the rover could be delivered to top of the Martian Atmosphere precisely.
The Mars 2020 team only needed to perform three of the possible six planned Trajectory Correction Maneuvers to hit their target. TCM-3 was performed on December 18th, 2020, 62 days before landing day and with about 78 million miles of distance left to travel.
Three days before landing, the team released the image below showing how well the group did at getting the spacecraft to the target at the top of the Martian atmosphere. This is what’s known as a B-Plane plot. You can think about this as a plane that goes through the center of Mars that is perpendicular to the velocity of the spacecraft.
On the plot, the green rectangle is the down-the-middle target the team was aiming for. At the top of the green rectangle are different colored ellipses with a ‘+’ in the middle of them. The + indicates the team’s best estimate of where the spacecraft would intercept the B-Plane, and the ellipses around the plus are noting where the team is predicating the spacecraft would intercept the B-Plane within a 98.89% probability. There are three different colored ellipses shown – each is a trajectory solution determined over the previous days.

There are a few things I’d like to point out here. First, the green down-the-middle target is approximately 15 km x 3 km; that’s small. Second, all of the ellipses are within the green rectangle; NASA’s team knew it was going to hit the small target. Third, engineers knew the spacecraft’s delivery point to less than 3 km, and fourth, the vehicle’s trajectory was determined to this precision when the distance between the spacecraft and Earth was about 115 million miles. Impressive doesn’t begin to cover it.
Atmospheric Braking and Guided Entry
Ditching the Cruise Stage
Having been delivered precisely to the top of the Martian atmosphere, it was time for the vehicle to start the process of landing. Ten minutes before the spacecraft hit the top of the Martian atmosphere, and about 17 minutes prior to the rover landing on the surface, the cruise stage was separated from the backshell and went on to burn up in the Martian atmosphere.
This was another step in solving the energy management problem that is landing a rover on the surface of mars. The cruise stage and its solar arrays, computers, propulsion and communication systems had done their jobs to bring the rover this far, and so there was no reason to bring all that mass safely to ground. Instead, the engineers cut it loose, and thus reduced the amount of energy needing to be managed in EDL by about 1.5 gigajoules (this is about equivalent to the kinetic energy of a Airbus A380 at cruising speed).
Creating Lift by Ejecting Some Mass and Steering To the Landing Target
About one minute after cruise stage separation, the spacecraft did one more thing that was crucial in solving the energy management problem of EDL: it ejected two pieces of dense tungsten called the cruise balance masses. Until this point in time, the center of mass of the cone shaped vehicle was located in the center of its geometry so that it could bbq-roll around its center-axis for thermal and stability reasons. After ejecting the cruise balance masses however, the vehicle’s center of mass was no longer in the center of the vehicle geometrically. The offset of the center of mass was done so that the vehicle had some lift during descent.
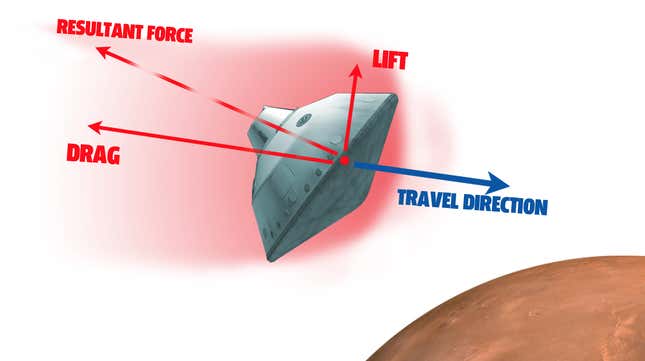
Ejecting the tungsten to throw off the vehicle’s center of mass was done to change the angle of attack and ultimately create the lift that was crucial in allowing the vehicle to bleed off its energy in just such a way that that energy would be zero just as the vehicle reached its landing target. Remember again that this is an energy management problem: if the vehicle doesn’t slow down fast enough, the rover could overshoot the landing site and/or crash. If instead the vehicle slows down too fast, it might fall out of the sky and not make it to the landing site at all.
During entry and descent, the vehicle slowed because of atmospheric drag (think of the force on your hand when you hold it out the window of your moving car). Atmospheric drag becomes stronger at a given velocity if the vehicle is running into more air. The air becomes exponentially denser (you can think of it as there being “more air”) the closer the vehicle is to the ground, so if the vehicle wants to slow down at a higher rate, it needs to descend faster. If the vehicle wants to slow down at a lower rate, it needs to descend slower. (Note drag is proportional to the density of the air and the square of the velocity). The image below is a vast oversimplification, but illustrates the point:
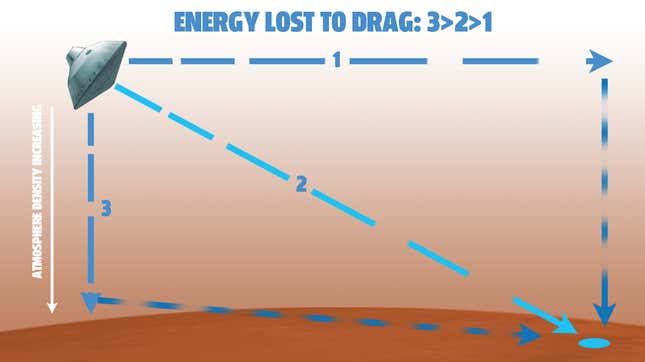
With the available lift vector provided by off-setting the center off mass, the vehicle now had some control over its rate of slowing and its direction of flight. It rolled the lift vector up, down, left, or right using thrusters on the top of the backshell (seen the four circles on the top of the backshell below. In this image, you can see the bottom left thruster firing). If it looked like the vehicle was slowing down too fast, it could roll to put the lift vector up thus staying in less dense air for longer, decelerating at a lower rate. If it looked like the vehicle isn’t slowing down fast enough, it could roll to put the lift vector down to find more air.
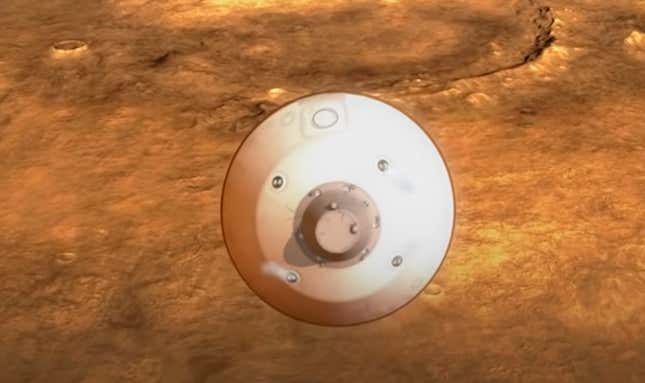
If the vehicle was descending at the desired rate, there was an option to put the lift vector to the left or right, allowing the vehicle to continue descending at its current rate. Banking to the left or right resulted in the vehicle flying away to the left or right from the landing site, however, so after some time flying to the right, the vehicle needed to roll the other way to correct the error. You may have heard this called out as ‘bank reversals’ if you were listening to the engineer’s call outs during EDL.
While this lift provided nothing like an airplane’s maneuverability, it was enough to allow the vehicle to accommodate the uncertainty of delivery and the uncertainty of the Martian atmosphere/weather while reducing the speed in just such a way that it’s zero when the rover makes it to the desired landing target.
Once the vehicle bled off the the excess energy and slowed down at the right rate, it could start flying directly to the landing site. In this phase of flight, the vehicle was descending much slower and staying about 10 miles above the surface. Again, you might have heard this being called out as “heading alignment” in the callouts from the engineering team.
The Heatshield
This slowing doesn’t come for free, however. Slamming into the atmosphere at 12,000 mph compresses the air in front of the vehicle causing significant heating of the thermal shield. Engineers calculated that the heatshield could see temperatures up to 3500 degrees Fahrenheit depending on the vehicle’s trajectory and atmospheric conditions.
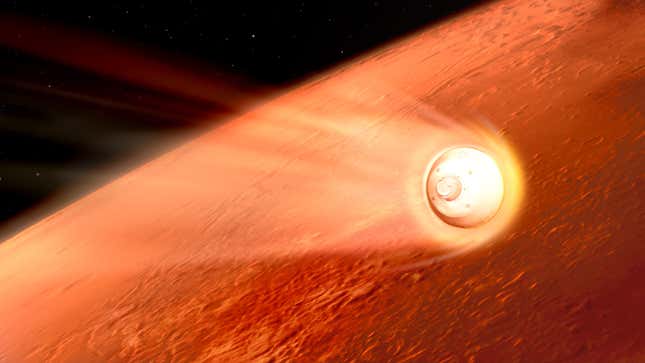
To protect the rover and the descent stage from temperatures hot enough to melt steel, the engineers designed a heatshield made of phenolic impregnated carbon ablator (PICA) that melts (or ablates) and takes heat away with it. Engineers estimated a reduction in heatshield thickness of up to 0.8 inches in some areas and about 125 pounds of total material loss due to the heat of entry. The heatshield was also thick and insulating which helped to keep the rover and the rover’s jet pack known as the descent stage safe inside.
Let’s Talk In Terms Of Energy
To assess how the vehicle performed during the actual landing in a bit more detail, I did some number-crunching using data shared via the live stream that showed some of the telemetry coming from the actual vehicle. This included the altitude, speed, and fuel used. For my math, I also used a simulation-based version of this display to get an estimate of downrange distance to Jezzero.
I calculated the vehicles potential and kinetic energy using the provided telemetry, the fuel remaining, and the mass of the different components listed on Wikipedia (I know this isn’t the best source, but I’ve checked them with some math based on other things I know about the vehicle’s performance and the mass of these components used for Curiosity. If they aren’t accurate, they’re at least close enough for what we’re using them for here).
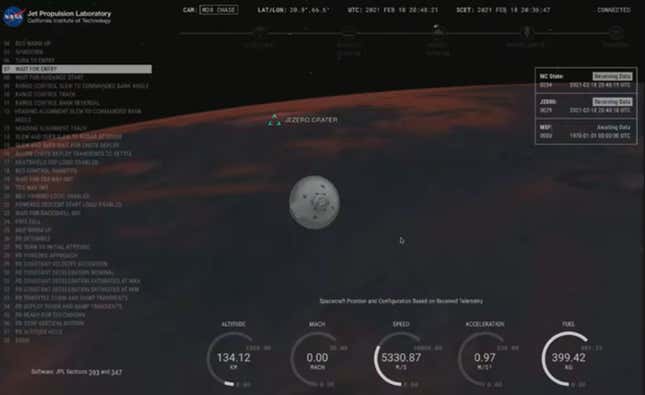
The vehicle hit the top of the atmosphere about seven minutes prior to landing, traveling in excess of 11,900 mph at an altitude of about 83 miles and a range (distance from the landing site) of about 395 miles. This gave the spacecraft a total energy of about 46 gigajoules.
One minute and 11 seconds later, the vehicle had completed its first bank reversal and was already at an altitude of 25 miles above the surface but had yet to reduce its speed with any significance. Twenty-six seconds later, the vehicle was at 13 miles altitude and started to impact much denser air, experiencing a peak of 10 Earth g’s of deceleration. One minute and 37 seconds after hitting the top of the atmosphere, the vehicle had cut its speed to almost half of what it was at entry, and energy was down to 30 percent of initial.
About four minutes after entering the atmosphere, the vehicle was eight miles above the surface of Mars and 12 miles from the targeted landing site. Using the atmosphere as a brake, the vehicle had removed almost 99 percent of the energy it came in with, but it was still traveling at over 1,000 mph. Now with the atmosphere only decelerating the vehicle at about a third of an Earth g, it was time to do something else.
Here’s a look at the changes in the speed, altitude, range to landing site, and potential and kinetic energy during the steps we’ve talked about so far. We’ll continue to look at this table as we talk through the rest of the steps of EDL.
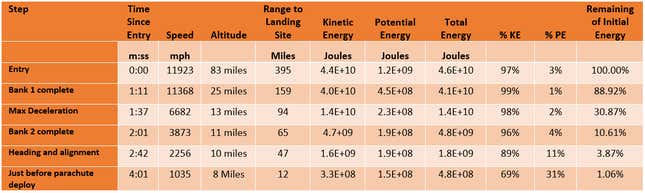
Deploying a Parachute at Over 1,000 mph
The next step in slowing down was to deploy the parachute once the vehicle had determined it was close enough to the landing target. The range to the target was estimated on-board by first knowing the vehicle’s starting position and velocity (sent to it by the engineers on Earth) and then, using a device to measure the acceleration and angular rate of the vehicle called an inertial measurement unit, calculating the change from that position to determine current location.
Before deploying the parachute, however, the vehicle first needed to get its center of mass re-aligned with its center axis to keep the vehicle stable when the parachute was deployed; deploying a parachute while going faster than the speed of sound is a violent thing to do to a valuable piece of hardware. Similar to how the center of mass was changed just prior to entry, the vehicle jettisoned six pieces of tungsten to re-center the craft geometrically. Ejecting these masses is known as the Straighten Up and Fly Right Maneuver.
With the mass now centered, the vehicle could deploy its parachute, but to deploy a parachute to slow a vehicle with a mass over 2600 kg and traveling roughly 1,000 mph was no small task. The parachute itself weighs about 150 lbs, was literally shot out of the vehicle at a speed of almost 100 mph, and needed to inflate to its 70 ft diameter in 0.5 seconds. Once inflated, the parachute, made of Technora, Kevlar, and nylon, pulled up on the vehicle at a neck-snapping 10 g’s and with nearly 60,000 lbs of stopping force.
Deploying a parachute at 1,000 mph on another planet is not something you can do with luck. It’s both an art and a science. I highly suggest watching the testing videos posted below to get a taste of what this takes.
Ditching The Heatshield
Twenty seconds after the parachute deployed, the vehicle was traveling less than 400 mph. At that speed, the heatshield was no longer required, so it was just extra mass that did not need to be safely landed on the surface. Separating the heatshield again shed energy. This time the reduction was about 2.6 megajoules of energy. That’s equivalent to the kinetic energy of an F150 traveling at 110 mph.
Separating the heatshield also served the more important purpose of letting the vehicle see the ground with its radar and camera system for the first time. Using the radar and camera system the vehicle could accurately determine its position and velocity relative to the surface of Mars.
The radar system hangs off the front of the descent stage. It sends microwave pulses at the ground and analyzes how those pulses are reflected back off the to determine height and velocity. The amount of time it takes for the pulses to return back to the radar system is an indication of distance to the ground below. By measuring the change in frequency of the return pulses, the vehicle could determine velocity using the Doppler Effect (remember that the radio signals have a frequency shift if the source is getting closer or farther away, and the amount of shift is dependent on speed).
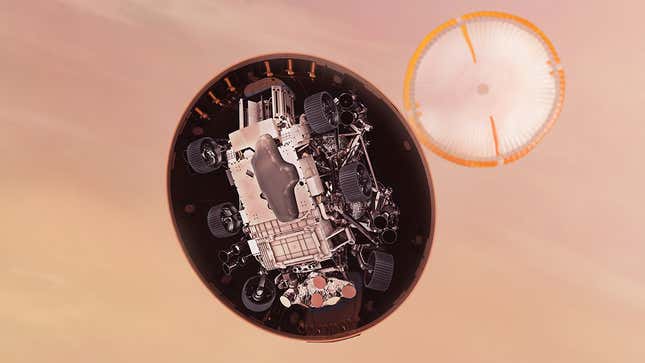
Using only the radar system and the inertial measurement unit, the vehicle was capable of determining its altitude to better than 330 ft of error, its speed to 5 mph, and its horizonal position to the nearest two miles. That’s not bad. In fact, it was good enough for the Curiosity rover to land in 2012, but given the tight constraints of Jezzero Crater, that just wasn’t going to cut it for Perseverance.
The Lander Vision System: Taking Photos Even When It Seems Impossible
To solve this problem, engineers developed the Lander Vision System (LVS), which consists of a camera placed on the side of the rover and software to perform Terrain Relative Navigation (TRN). This system took pictures of the surface of Mars, analyzed those images to find landmarks, and then matched those up to an on-board map to determine the vehicle’s location to better than 57 feet. This is a significant upgrade over what Curiosity was capable of in 2012.
Especially impressive was that this system was capable of taking clean enough images of the surface while moving at hundreds of miles-per-hour and jostling around at the end of a parachute. According to a paper written in 2017, the LVS system was designed to determine the vehicle’s position accurately even if swinging at 50 degrees/second and looking 45 degrees away from the ground directly below. That’s incrdible.
One minute and 10 seconds after heatshield separation, both the radar and LVS had worked together to determine the vehicle’s position and velocity to much higher precision. The parachute had also stopped nearly all of the vehicle’s horizonal velocity, and the landing target was almost directly below.
The vehicle had slowed down an additional 800 mph since deploying the parachute, but after about one minute and 45 seconds of using the parachute, the vehicle had reached its terminal velocity. Due to the thin atmosphere on Mars, even with a parachute with the volume of a large house, the vehicle was still traveling around 185 mph. With the ground two miles below the vehicle, this meant that if nothing further had been done to slow the rover, the vehicle would have impacted the surface in about 40 seconds. It was time to use rockets.

Powered Descent and the Skycrane Maneuver
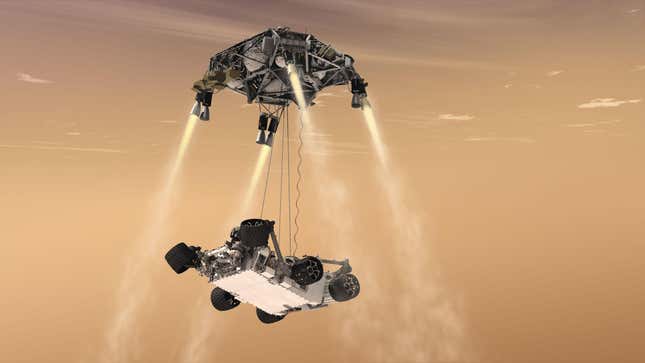
One minute prior to landing, the rover and the descent stage separated from the backshell (the top part of the shell that protected the rover and descent stage during atmospheric entry) and the parachute. Again, this was done to discard mass and limit the amount of energy that needed to be bled off in the next phase of flight.
Before starting to use the thrusters, during the 2.4 seconds of free fall, Perseverance chose precisely where to land based on the current information. EDL systems engineer Erisa Stilley explained: “Once Perseverance has a better sense of where she’s at, she then uses a second onboard map, based on where we can currently divert to in that point in time, and searches that area to find the safest place she can fly to.”
The Soon-To-Be-Crashed Descent Stage Fires Up Its Thrusters
After falling away from the backshell, the descent stage fired up its eight thrusters, each capable of throttling between 90 and 670 pounds of thrust. Using the 400 kg of the colorless, flammable hydrazine fuel onboard, the descent stage took the vehicle to the selected landing site. Before heading to the landing site, however, the vehicle performed a divert maneuver so that it wouldn’t risk running into the backshell and parachute it had just separated from.
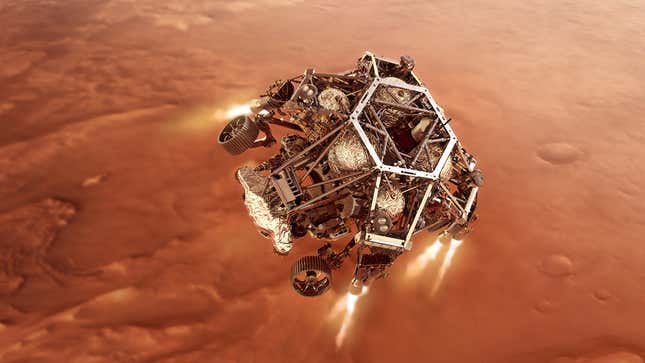
To steer the vehicle away, the stationary thrusters were throttled to vary their force output. Creating a higher force on the left side of the vehicle than on the right caused the vehicle to rotate clockwise. Once tilted slightly to the right, the thrust could steer the vehicle to the right while still slowing the vehicle’s descent to the ground.
During the final minute of flight, the vehicle was constantly taking measurements to determine its position, orientation, and velocity and then using that information to inform how to throttle thrusters to get safely to the desired landing spot. This loop of measurement, calculation, and commanding was done many times a second and getting this intricate dance wrong, even for just a second, could have caused the vehicle to crash.
Once the parachute and backshell were avoided, the vehicle maneuvered itself over the desired landing spot, nulled its horizonal velocity, and came in directly above the landing site.
Why NASA Lowered The Rover With Cables
When the rover was 69 feet above the ground, half the engines turned off and the remaining thrusters were throttled so the vehicle could descend to the surface at a constant velocity of 1.7 mph.
When the descent stage was 65 feet above the surface, the rover was lowered down on 20ft long cables. This was done for a few reasons. First, so that the thrusters could remain a safe distance from the ground and not kick the dust on the surface at a speed that could damage the rover. Second, if the rover instead sat on a platform with thrusters, it would be top heavy and possibly roll over if the vehicle landed on a slight hill or a rock. Lowering the vehicle directly onto its wheels allowed the team to take advantage of the rovers off-road capability: anything the rover could drive on, it could land on.
Immediately after sensing that the rover had touched down (called “tango delta” by the operations team) on the surface, the descent stage cut that cables it had lowered the rover on, throttled up its thrusters, and used the remaining fuel to get as far away from the rover as it could before crashing into the surface.
All told, EDL had lasted seven minutes. It slowed the rover from almost 12,000mph, bled off 46 gigajoules of total energy, and placed Perseverance safely on the surface of Mars within the landing target of Jezero Crater and within five meters of the landing target selected at backshell separation.
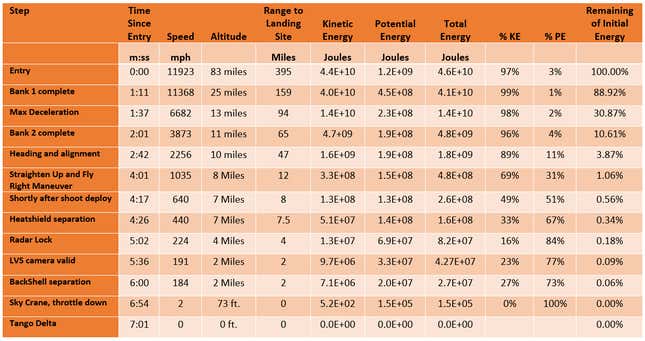
Media Released Since Landing
Since Perseverance landed safely on February 18th, NASA has released a treasure trove of images and video depicting how well EDL went, and now with a deeper level of understanding of what it took to accomplish this, I hope you can enjoy them just a little bit more.
First up, NASA released this image taken by the Mars Reconnaissance Orbiter’s HiRISE Imager of Perseverance descending on its parachute. We’ve discussed in detail what it takes to understand where a spacecraft is and how to get it to where you want it to be. Now imagine doing it with two spacecraft and having one take a picture of the other. Truly amazing.
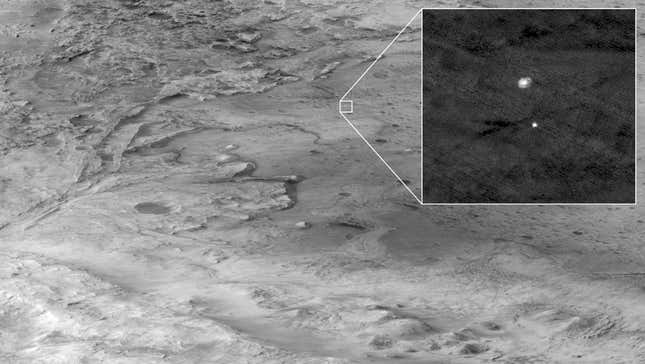
After Perseverance landed, the Mars Reconnaissance Orbiter also managed to take this picture showing where everything landed. Here you can see that the parachute and backshell landing about 1.2 km from Perseverance while the heat shield ended up about 1.5 km from Perseverance. Using the fuel remaining after placing the rover on the surface, the descent stage managed to get 700 meters from the rover before crashing.
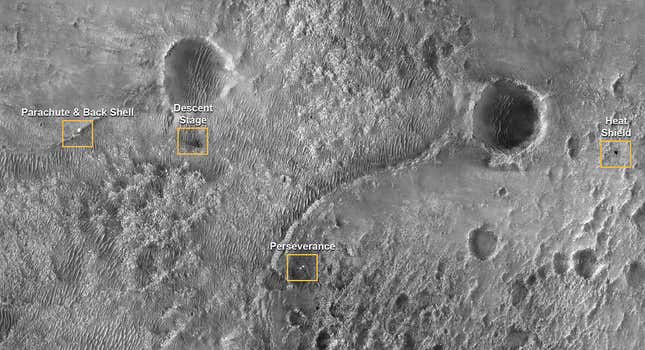
Speaking of the descent stage, the rover managed to capture a picture of the smoke plume created when it crashed into the surface.
Dare Mighty Things
But the crème de la crème of all the material released is the video taken by vehicle itself during the actual Entry Descent and Landing. Armed with the information discussed in this article, see if you can follow along.
As you watch that, think about what it took just to get the vehicle to Mars to even attempt a landing and the incredible precision with which the vehicle was delivered to the top of the atmosphere. Marvel at the immense energy that was bled off into heat and sound as the vehicle descended through the atmosphere, and that the energy was bled off in a controlled fashion so that the vehicle’s speed would be almost zero just as the rover reached its landing target.
Appreciate how the violent parachute deployment at over 1,000 mph looked so effortless and was set up for success by ejecting six ballast masses just before parachute deployment in what is called the straighten up and fly right maneuver. Nod with understanding as the heatshield separates from the rover to reduce the remaining energy needing to be bled off before landing.
Watch as the rover swings from the parachute as the radar and Lander Vision System (LVS) determine precisely where the spacecraft is and just how fast it’s moving relative to the Martian surface. Enjoy watching the vehicle divert on thrusters away from the parachute and then maneuver directly over the chosen safe landing target.
Notice as the descent stage slows to a constant speed of under two mph as it nears the surface, and see if you can spot in the stirred up dust exactly when the vehicle switched from eight thrusters to four.
Gawk as the vehicle is lowered by the descent stage to the surface on cables, is engulfed in dust kicked up by thrusters, and safely touches down on the surface of Mars.
Most importantly, view the video with the appreciation and knowledge of how difficult the landing was to accomplish and what it says about what people are capable of if they come together and, as NASA’s Jet Propulsion Lab would say, “Dare mighty things.”